Synthetic biology, along with CRISPR-Cas9 and genome editing, has become one of the popular buzzwords in the life sciences sphere over the past 10 years, but what exactly is it?
Synthetic biology (or affectionally known as ‘synbio’) is a marriage between engineering and biology, whereby the principles of engineering are applied to biological systems and organisms. The whole aim of synbio is to modify existing systems as well as create new ones in order to solve a variety of problems. Plus, it is also used just to have a bit of fun (as you’ll soon find out!).
On with the basics
The basic principles of engineering are abstraction, standardisation, and quality control.
Abstraction
Abstraction basically means you don’t have to understand how every component of a system works before you actually get to put the system into practice. This is similar to the way we use computers in our everyday lives without knowing exactly how all the component parts communicate with each other at a technical level (though some of us might!).
When applied to biology, this means that scientists don’t necessarily need to know the exact DNA code if they intend to design a synthetic biology device/system. This is because DNA sequences have been standardised into synbio ‘parts’ that can be combined to create ‘devices’, which in turn are further combined to create systems:
DNA —> Parts —> Devices —> Systems
Examples:
Parts: Promoter, protein coding sequence, terminator (each one is a part)
Device: A plasmid which encodes a protein
System: A biosensor which works via multiple interacting proteins
This will become clearer when we look at a few examples of synthetic biology in use.
Standardisation
This leads us to standardisation. Standardisation of synbio parts ensures that these parts are reusable and interoperable (i.e. can be used in various situations and have the same function). Standardisation also leads to easier scalability and production.
One popular synbio standard is the BioBrick, which is used in the popular synbio competition, iGEM. BioBrick parts have a prefix and suffix sequence attached to the DNA sequence of interest. The prefix and suffix contain restriction sites (i.e. specific sequences where restriction enzymes can cleave the DNA). Since the prefix and suffix are standardised, it is possible to have multiple iterations of BioBrick assembly, and hence more complex ‘devices’ can be built.
Engineering biology: the stages of development
In engineering biology, there are some key stages for the development of a complete system/device:
Characterisation —> Automation —> Optimisation —> Industrialisation
Let’s walk through them step-by-step.
When we talk about characterisation, the key questions we ask are: what parts do we need? Which version of each part is the most optimal (e.g. promoters, gene sequence, terminator)? We want to know what parts will help us create the desired system, and certain parts work better together than others – hence it is critical to compare and contrast various combinations, and if necessary, test them.
After the parts are characterised, the building process can be automated using robotic arms and other specialised machinery like multi-channel pipette systems. This process is usually done in specialised labs called ‘foundries’. Moving forward, we need to ask ourselves which combination of parts would be the best option, as part of the optimization step. And finally, industrialisation involves ensuring the process is reproducible and reliable. It is pretty much the stage where large-scale manufacturing takes place.
All these principles are the sub-tenets of the synthetic biology ‘dogma’, which is also known as the design-build-test-learn cycle (See Figure 1).

Figure 1: An overview of the Design-Build-Test-Learn (DBTL) cycle.[Link to source].
Why should we care about all this stuff?
What can synbio actually help us do?
The short answer is, quite a lot.
Synthetic biology can potentially be used to improve agriculture, produce chemicals and biologics, generate bioenergy, make synthetic animal products like fake meat or milk, as well as produce new vaccines and drugs.
On the academic side, synbio is used to construct a variety of circuits and systems and reconstruct or synthesise whole genomes. These exercises are more for simply seeing how far the technology can go, which thereby tests its biological limits rather than for a specific application.
One very common application of synthetic biology is for the creation of biosensors. These are devices which detect a certain characteristic (like blood sugar, for example) by combining a biological component with a detector component. A pulse oximeter is a good example of a biosensor — it detects blood oxygen saturation and pulse rate.
In a synbio biosensor, most components are made up of biological parts (usually genetic circuits) which function together as a unit. They usually use reporter molecules like fluorescent proteins for detection.
In general, the applications of biosensors can extend to:
Solving environmental issues — detect pollutants and heavy metals
Monitoring diseases — by acting as internal or external monitors of our health conditions
Pathogens detection — for instances, the presence of parasites and/or its infection could be discovered
A short case study
A classic example is an arsenic biosensor which can detect dangerous amounts of arsenic in water. You would ideally need a cheap, accurate, safe, and easily readable sensor that can be used in field conditions (like in rural areas). The researchers decided to modify the natural arsenic detoxification system in E. coli to act as a sensor.
Here’s what the circuit looks like [Source]:

Figure 2: A schematic of an engineered E. coli arsenic detoxification system.
This looks complicated at first, but if you break it down into smaller parts - it becomes very clear. Let’s look at each part and what they do:
ArsR — acts as the repressor that binds to the ParsR promoter in the absence of arsenic
ArsC — works as the arsenate reductase, meaning that it catalyses the conversion of arsenic ions to arsenite ions
GFP — stands for the green fluorescent protein, which is the reporter molecule
In the absence of arsenic, the ArsR protein binds to its own promoter and inhibits the production of GFP.
On the other hand, when arsenic is present, ArsC converts As5+ (arsenic) into As3+ (arsenite). Arsenite (red dots) then binds to ArsR, causing it to unbind from the ParsR promoter. Now the promoter is free for RNA polymerase to bind and transcribe the gfp gene. RNA polymerase is a protein which transcribes DNA into RNA, which can then be translated into the corresponding protein by the ribosome.
This is all engineered into E. coli, which is generally a very reliable and useful chassis for synthetic biology, because it has a lot of tools specifically developed for it.
More applications
Biosensors are a more useful application of synbio, but what about the fun stuff? A particularly cool application of synbio is bacterial photography — which is a way of programming bacteria to ‘see’ light. This uses concepts from optogenetics, which is all about using light to control the expression of genes.
To do this, scientists fused a light-sensing protein domain (for instance, PCB, which stands for the phytochrome) to a signalling domain called a histidine kinase domain (which is taken from E. coli). The PCB detects the presence of light, and the histidine kinase catalyses a signal cascade which then results in the transcription of a downstream gene.
This is a chimera between a plant and a bacterial system. It works in a slightly counterintuitive manner – in the presence of light, the histidine kinase is inactive as there is no phosphorylation; whereas in the absence of light, the kinase domain becomes phosphorylated (meaning that phosphate is added covalently), and downstream signalling can thus occur.
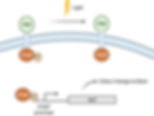
Figure 3: Engineered system for bacterial photography. This figure was adapted from Levskaya et al. ‘s Engineering Escherichia coli to see light (2005).
The phosphorylated OmpR (which has the histidine kinase domain) activates the ompC promoter. lacZ is the reporter gene that codes for β-galactosidase. This β-galactosidase would be responsible for breaking down a dye called S-gal, resulting in a colour switch to black.

Figure 4: An example of a ‘bacterial photograph’ [Source: In supplementary material]
In the areas of light, no downstream signalling occurs, and hence lacZ does not get transcribed. So, there will not be any colour changes to black. In contrast, when light is absent, downstream signalling occurs and the dye turns black in colour (as seen in the darker areas of the bacterial photograph).
This is just one of many possible applications of optogenetics. Interestingly, optogenetics has also been used to control behaviour in mice. By modifying the mouse embryo to express a light-activated ion channel on its nerve cells, neuronal signalling can be induced by an external light source. Ion channels have to open and close to allow transmission of nerve signals, and hence controlling them allows us to control the signal.

Figure 5: Close up schematic of the optogenetic system used to control behaviour in mice [Source]. Figure created on BioRender.
Check out this YouTube video if you want to learn more: https://www.youtube.com/watch?v=v7uRFVR9BPU
The ethical dilemma of synthetic biology…
The above experiment, although very interesting, has serious ethical implications. What if we were to modify a human embryo to do the same thing? Where is the limit? One could argue that the findings of these experiments would lead to breakthroughs in disease treatment (e.g. neurological disorders, Parkinson’s, etc.), however each stage of experimentation must be ethically justified before it can be carried out, and there are specific rules and regulations in place to ensure this. Before experiments can be approved, they must pass an ethics board review by providing justifications as to why the research is necessary and why it must be done by so-and-so particular method. This ensures that scientists have done their due diligence and understand the implications of their work before carrying it out.
Synthetic biology has many exciting real-world applications from improving agriculture to producing new medicines. One particularly inspiring innovation is synthetic milk – wherein the molecular components of cow’s milk (whey, casein, etc.) are produced in engineered cells and mixed to produce a pseudo-milk which is nutritionally the same as cow’s milk. This seems like a stellar idea, but we must think of the potential socio-economic and political implications of such technology. For example, this could affect farmers’ livelihoods as demand for cow milk would be replaced with synthetic milk, however, it could also benefit the environment as it requires less energy and carbon emissions than dairy farming.
Technology can have unforeseen consequences, and science is not done in isolation from politics and socioeconomics. Not everyone is affected in the same way by innovations (as in the example of the farmers losing their income), so it is important to allocate responsibility and accountability for these decisions.
So, whether it is biosensors, synthetic milk, or bacterial photographs, with great power comes great responsibility, and we must be vigilant and mindful of the real-world effects of research at every stage.
Author
Devyani Saini
BSc Biochemistry
Imperial College London
Disclaimer: All figures created using BioRender are intended solely for educational purposes and not for profit.